Gregarina
Gregarines
Sonja I. Rueckert and Brian S. Leander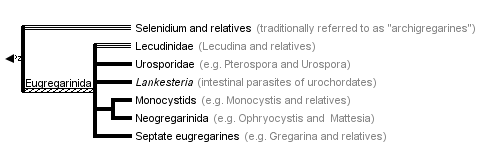


This tree diagram shows the relationships between several groups of organisms.
The root of the current tree connects the organisms featured in this tree to their containing group and the rest of the Tree of Life. The basal branching point in the tree represents the ancestor of the other groups in the tree. This ancestor diversified over time into several descendent subgroups, which are represented as internal nodes and terminal taxa to the right.
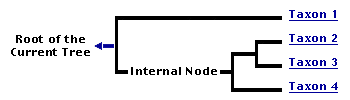
You can click on the root to travel down the Tree of Life all the way to the root of all Life, and you can click on the names of descendent subgroups to travel up the Tree of Life all the way to individual species.
For more information on ToL tree formatting, please see Interpreting the Tree or Classification. To learn more about phylogenetic trees, please visit our Phylogenetic Biology pages.
close boxIntroduction
Gregarines are a diverse group of apicomplexan parasites that inhabit the intestines, coeloms and reproductive vesicles of marine, freshwater and terrestrial invertebrates. Approximately 250 genera and 1650 species have been described so far (Clopton 2000; Hausmann et al. 2003; Levine 1976, 1977, 1988). Some ancestral characters found in gregarines (e.g. extracellular feeding stages and a monoxenous life-cycle) have given the group a reputation of being “primitive”. Although some lineages of gregarines have retained characters inferred to be ancestral for the group, and perhaps apicomplexans as a whole, most gregarines represent highly derived parasites with novel ultrastructural and behavioural adaptations (Leander 2008). Many marine gregarines, for instance, have become giants among single-celled organisms and have evolved diverse patterns of surface structures (Fig. 1).

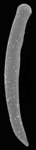
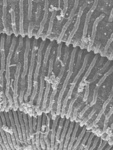
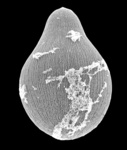
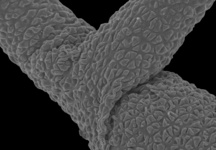
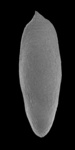
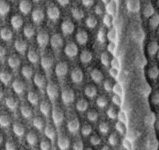
Fig 1. Scanning electron micrographs of different marine gregarines; from left to right: Selenidium, surface of Selenidium (high magnification), Lecudina, surface of Pterospora (high magnification), Lankesteria and surface of Lankesteria (high magnification). (© Sonja I. Rueckert/Brian S. Leander)
Comparative gregarine cell morphology placed in a modern phylogenetic context helps clarify the earliest stages of apicomplexan evolution and demonstrates novel ways in which these parasites have solved fundamental biological problems, such as locomotion (nematode-like bending movements, gliding motility and peristalsis), host-dependent nutrition (myzocytosis, nutrition absorption via the surface) and reproduction. The overall biodiversity and phylogenetic relationships of gregarines are very poorly understood and only a small minority of the (predicted) total number of extant gregarine species have been described so far. Less than 1% of known invertebrates have been examined for gregarine infection (Clopton 2000). A compounding issue is that only a tiny number of described gregarine species have been studied at the molecular level, which makes species delimitation (using molecular barcodes) and inferences about broader phylogenetic relationships among gregarines very difficult.
Characteristics
Gregarines are single-celled apicomplexan parasites of invertebrates; some gregarines species might be commensals. The group is characterised by the following general features:
- Apical complex in the sporozoite stage; lost in the trophozoite stage in eugregarines and neogregarines
- Attachment to host via a mucron (in aseptate gregarines) or an epimerite (in septate gregarines); some gregarines (e.g. urosporidians) float freely within extracellular body cavities (e.g. coelom)
- Relatively large spindle-shaped cells, compared to other apicomplexans and eukaryotes in general (some over 850 µm in length)
- Tophozoite cell shapes very diverse in dfferent species
- Monoxenous life-cycle, requiring only one host (nearly all species)
- Inhabit extracellular body cavities of invertebrates such as the intestines, coeloms and reproductive vesicles
- Diverse modes of locomotion (e.g. gliding, bending and peristalsis)
- Different modes of feeding (e.g. myzocytosis and surface mediated nutrition)
- Most gregarines with longitudinal epicytic folds; crenulations in urosporidians
- Paraglycogen storage products give gregarine trophozoites a brownish colour
- Mitochondria with tubular cristae, often distributed near the cell periphery
- Syzygy—the process in which two mature trophozoites pair up before the formation of a gametocyst
- Large conspicuous nucleus (and nucleolus) in the trophozoites
Hosts
Gregarines inhabit different body spaces within the invertebrate host, such as the intestines, coeloms and reproductive vesicles. Most of the species are known to be host specific: Selenidium spp. infect polychaetes and sipunculids; Lankesteria spp. infect the intestines of ascidians; Monocystis spp. infect the reproductive vesicles of earthworms; neogregarinida infect the cuticle of insects, such as butterflies (Ophryocystis on the Monarch Butterfly); septate gregarines infect the intestines of marine and terrestrial arthropods.

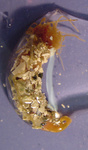
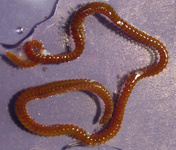
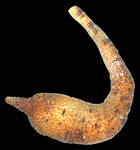
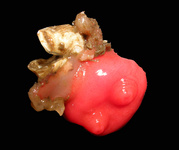
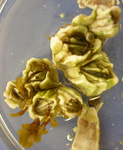
Fig. 2. Light micrographs of a few marine invertebrates known to be infected with intestinal gregarines; from left to right: Thelepus (Polychaetes), Lumbrinereis (Polychaetes), Phascolosoma (Sipuncula), Cnemidocarpa (Urochordata) and Balanus (Crustacea). (© Sonja I. Rueckert/Brian S. Leander)
Classification
Traditionally, three general categories have been used to lump gregarines based on habitat, host range and morphological features of the trophozoites: archigregarines, eugregarines and neogregarines.
As the term archigregarines implies, some members of the group (e.g. Selenidium) have retained several ancestral features, such as exclusive presence in marine habitats and the presence of intestinal trophozoites that are similar in overall morphology to the infective sporozoites. They also have 0-50 longitudinal epicytic folds, bundles of microtubules beneath the cell surface and nematode-like bending behaviour (compare Grassé 1953, Schrével 1971a, b).
Eugregarines are found in marine, freshwater and terrestrial habitats and possess large trophozoites that are significantly different in morphology and behaviour from the sporozoite stage. The trophozoites have lost the apical complex and instead possess a mucron (or an epimerite). The surface is inscribed by 90-300 epicytic folds, resulting in stiff cells that are capable of gliding motility. Intestinal eugregarines are separated into septate (mostly terrestrial, like Gregarina) and aspetate (mostly marine like the Lecudinidae and Urosporidae) gregarines, depending on whether the trophozoite cell is superficially marked by a transverse septum (Fig. 3). Urosporidians (e.g. Pterospora) are aseptate eugregarines that infect the coelomic spaces of marine polychaetes. Monocystids are aseptate eugregarines that infect the reproductive vesicles of terrestrial annelids and tend to branch closely with neogregarines in phylogenetic trees.
Neogregarines are found in terrestrial hosts (e.g. insects); they have reduced trophozoite stages and, like monocystids, tend to infect host tissues other than the intestines (Leander 2008).
General Life Cycle
The transmission of gregarines to new hosts usually takes place by oral ingestion of oocysts in both aquatic and terrestrial environments. Some gregarine oocysts might be transmitted with host gametes during copulation (e.g. Monocystis, see Fig. 5). In either case, four or more sporozoites (depending on the species) equipped with an apical complex eventually escape from the oocysts (Fig. 4, steps 8 and 1), find their way to the appropriate body cavity and penetrate the host cells. The sporozoites emerge from a host cell, begin to feed and develop into larger trophozoites (Fig. 4, step 2). Some gregarines have sporozoites and trophozoites that are capable of asexual replication, a process called schizogony (or merogony); most gregarines appear to lack schizogony in their life-cycles (or at least this process has yet to be observed).
Two mature trophozoites eventually pair up in a process called syzygy and develop into gamonts (Fig. 4, step 3). The orientation of gamonts during syzygy differs depending on the species (e.g. side-to-side and head-to-tail). A gametocyst wall forms around each pair of gamonts (Fig. 3, step 4), which then begin to divide into hundreds of gametes (Fig. 4, step 5). This process is called gametogeny (Fig. 4, process B). Pairs of gametes fuse and form zygotes (Fig. 4, step 6), each of which becomes surrounded by an oocyst wall (Fig. 4, step 7). Within the oocyst, meiosis occurs to yield four or more spindle-shaped sporozoites (Fig. 4, process A = sporogony) (Kuriyama et al. 2005). Hundreds of oocysts accumulate within each gametocyst, and are usually released via host feces or via host death and decay.

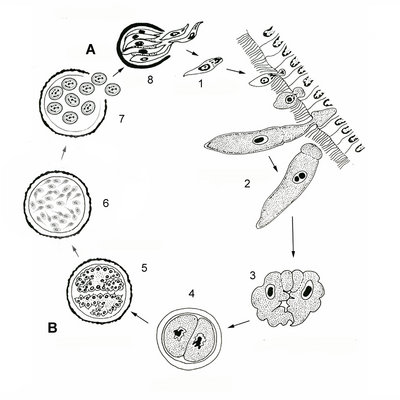
Fig. 4. Illustration of the general life cycle of gregarines: A. Spindle-shaped sporozoites are produced in the oocyst via meiosis (sporogony); B. Inside the gametocyst gamonts divide into hundreds of gametes (gametogeny); see text above for a detailed description. © Sonja I. Rueckert (modified after Vivier & Desportes 1990)
Specific Examples of Gregarine Life Cycles
Monocystis
Fig. 5 illustrates the life cycle of Monocystis agilis. The infection starts with the hermaphroditic earthworm host ingesting an oocyst (1, 2) that releases sporozoites (3, 4) within the intestines (green). The sporozoite is motile and penetrates the intestinal wall (5) and enters the dorsal blood vessel (6). The sporozoite enters the seminal vesicles (yellow) via the (dark red) dorsoventral hearts (7). The sporozoites feed on the host's developing spermatocytes (8) in the wall of the seminal vesicle. The gregarines then move into the lumen of the vesicle where they mature into trophozoites. Each ball of sperm within the seminal vesicle contains a young trophozoite (9). These trophozoites are covered by remnants of the sperm cells and often superficially take on the appearance of a ciliated eukaryotic cell. After consuming the sperm ball, the now mature trophozoites pair up in syzygy (10). The trophozoites develop into gamonts, and a gametocyst wall forms around each pair (11). Each of the two gamonts undergoes multiple nuclear divisions to produce many nuclei developing into gametes. Two gametes each fuse and form zygotes, which are surrounded by oocyst walls (12). Within this oocyst, the diploid zygote undergoes meiosis and then mitosis to produce eight haploid daughter cells by a process known as sporogony. The gametocysts, or oocysts if they have been released, leave the earthworm through the male genital pore and are liberated into the soil (13, 14). Infection of a new host occurs by oral ingestion of an oocyst (or perhaps through the process of mutual cross fertilization during host sexual reproduction).

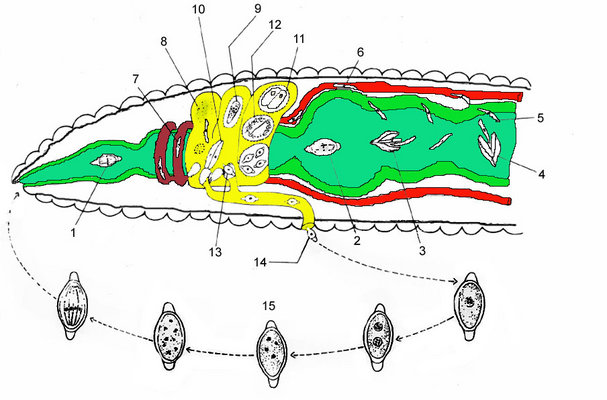
Fig. 5. An illustration of the Monocystis life cycle. The infection starts with an earthworm ingesting an oocyst, and the parasites then develop within the host's seminal vesicle; see text above for a detailed description. © Sonja I. Rueckert (modified after Olsen 1974)
Ophryocystis elektroscirrha
The life cycle of Ophryocystis elektroscirrha is closely correlated with that of its host the monarch butterfly (Danaus plexippus). Parasite spores are transmitted maternally during oviposition, when infected females scatter spores over eggs and plant (i.e. milkweed) surfaces (McLaughlin & Myers 1970). Spores can also be transferred horizontally between adults during mating or other incidental contact. However, the ingestion of the spores by larvae is necessary to start a new infection. Within the larval gut, the spores lyse and release sporozoites that penetrate the intestinal wall, enter the hypoderm and undergo two vegetative, asexual replications. During this time, the monarch larvae pass through five developmental instars (compare Altizer & Oberhauser 1999). After pupation of the host, the parasite completes a sexual reproduction cycle, which ends with the formation of dormant spores on the scales of the developing adult butterfly (McLaughlin & Myers 1970). The density of the spores is highest on the abdomen, but spores can also be found on the wings, head and thorax (Leong et al. 1992).
Heavily infected adults have difficulty emerging from the pupal cases and expanding their wings; butterflies with low parasite infections appear to be normal (McLaughlin & Myers 1970, Leong et al. 1992). Ophryocystis elektroscirrha compromises the fitness of monarch butterflies by affecting survival to eclosion, adult size, lifespan, and male reproductive success. These effects are dependent on the parasite load of the butterflies and are mainly restricted to heavily infected individuals (see Altizer & Oberhauser 1999).
Motility
Gregarines have adopted different modes of motility. The trophozoites of Selenidium species, for example, are able to bend and coil; see movies:
View Selenidium terebellae movement (from the intestines of a spaghetti worm, Thelepus)
-
View Selenidium vivax movement (from the intestines of a peanut worm, Phascolosoma) (Leander 2006)
One or more layers of microtubules are known to be responsible for these nematode-like movements (Leander 2007, Schrével 1971a, Stebbings et al. 1974). Within the eugregarines, the number of epicytic folds has increased in some lineages (revisit the SEM of Lecudina in Fig. 1) leading to relatively stiff cells and a distinctive gliding motility; see movie:
-
View Lecudina sp. gliding (from the intestines of the pile worm Nereis) (Leander 2008).
The epicytic folds of some eugregarines are capable of metachronal undulations. This behaviour was initially interpreted as the driving force behind gliding motility (Vivier 1968, Vavra & Small 1969). Nonetheless, a study has subsequently shown that the gliding motility is facilitated by an actinomyosin system organized beneath the longitudinal edge of each epicytic fold (Heintzelmann 2004). In addition to these two types of motility, dynamic peristalsis-like movements can be found in the urosporidians and some archigregarines; see movies:
-
View Pterospora schizosoma dynamic peristalsis-like movements (a urosporidian from the coelom of a bamboo worm, Axiothella) (Landers & Leander 2005)
View Selenidium vivax dynamic peristalsis-like movements (from the intestines of a peanut worm, Phascolosoma) (Leander 2006)
These dynamic cell behaviours are thought to facilitate the acquisition of nutrients from the surrounding medium through micropores in the cell surface of the trophozoites.
Apicoplast?
The apicoplast is a remnant, non-photosynthetic homologue to chloroplasts found in many apicomplexan parasites. This organelle was observed first in the 1960s by transmission electron microscopy, but was not identified as a plastid until a 35 kb plastid genome was characterized in 1996 (McFadden et al. 1997, Waller & McFadden 2005; Wilson et al. 1996, Wilson & Williamson 1997). The apicoplast is surrounded by four membranes and has been found in nearly all major groups of apicomplexan parasites (Waller & McFadden 2005). This organelle is inferred to be an ancient feature of the group that was acquired by secondary endosymbiosis with a red algal prey cell. However, apicoplasts appear to be absent altogether in Cryptosporidium (Zhu et al. 2000, Abrahamsen et al. 2004, Xu et al. 2004).
The only evidence for the possible existence of an apicoplast in gregarines is a transmission electron micrograph from Selenidium pendula by Schrével (1971b). Schrével (1971b) found conspicuous vacuoles that were surrounded by four membranes; these structures were unlike any other structure described in gregarines. At that time, apicoplasts had not been discovered. Toso & Omoto (2007) showed by a number of criteria that G. niphandrodes does not have a plastid or plastid genome. Therefore, it remains undetermined whether or not any or all gregarines possess an apicoplast.
Discussion of Phylogenetic Relationships
Most studies on the phylogenetic relationships of gregarines are based on small-subunit ribosomal DNA sequences from a few species (Carreno et al. 1999, Leander 2007, Leander et al. 2003a, b, Leander et al. 2006, Roychoudhury 2007, Rueckert & Leander 2008, Valles & Pereira 2003). Although comparative morphology indicates that gregarines are monophyletic, whether or not other apicomplexan lineages like Cryptosporidium and coccidians sensu stricto are nested within them remains to be rigorously tested with molecular phylogenetic data.
Comparative morphology and molecular phylogenetic data strongly suggest that Selenidium (and relatives) forms a paraphyletic group from within which eugregarines and some other highly derived lineages evolved (e.g. Selenidium vivax and relatives) (Leander 2008).
The Eugregarinida contains most of the known gregarine species. They have elaborate systems of epicytic folds, with an actinomyosin-based gliding apparatus. The apical complex has been lost in the trophozoites, but an attachment structure is present (e.g. a mucron or an epimerite). The phylogenetic relationships within the Eugregarinida are just beginning to be addressed with molecular phylogenetic approaches and our understanding of the group's biodiversity and interrelationhsips is still very limited:
- Archigregarines, such as Selenidium, are paraphyletic
- The monophyly and composition of the Eugregarinida are uncertain
- Lecudina is paraphyletic
- Lankesteria is monophyletic and is most closely related to lineages of Lecudina
- Urosporidians (e.g. Pterospora) evolved from within the Lecudinidae
- Septate eugregarines (e.g. Gregarina and Leidyana) that infect insects are monophyletic
- Monocystids (e.g Monocystis) form a sister group to neogregarines (e.g. Ophryocystis and Mattesia)
- Gregarine SSU rDNA sequences tend to be highly divergent and form long branches in molecular phylogenetic analyses
- At this point, only 25 gregarine species have a published SSU rDNA sequence
- The vast majority of gregarine species have never been studied at the molecular level
References
Abrahamsen, M., Templeton, T., Enomoto, S., Abrahante, J.,Zhu, G., Lancto, C., Deng, M., Liu, C., Widmer, G., Tzipori, S., Buck, G., Xu, P., Bankier, A., Dear, P., Konfortov, B., Spriggs, H., Iyer, L., Anantharaman, V., Aravind, L., and V. Kapur. 2004. Complete genome sequence of the apicomplexan, Cryptospridium parvum. Science 304: 441-445.
Altizer, S., and K. Oberhauser. 1999. Effects of the protozoan parasite, Ophryocystis electroscirrha, on the fitness of monarch butterflies (Danaus plexippus). Journal of Invertebrate Pathology 74: 76-88.
Carreno, R. A., Martin, D. S., and J. R. Barta. 1999. Cryptosporidium is more closely related to the gregarines than to coccidia as shown by phylogenetic analysis of apicomplexan parasites inferred using small-subunit ribosomal RNA gene sequences. Parasitology Research 85 (11): 899-904.
Clopton, R. E. 2000. Order Eugregarinorida Léger, 1900. Pp. 205-298 in J. J. Lee, G. F. Leedale, and P. Bradbury, eds. The illustrated guide to the Protozoa 2nd Edition. Allen Press Inc., Lawrence.
Coulon, P., and M. Jangoux. 1987. Gregarine species (Apicomplexa) parasitic in the burrowing echinoid Echinocardium cordatum: occurrence and host reaction. Diseases of Aquatic Organisms 2: 135–145.
Grassé, P.-P. 1953. Classe des grégarinomorphes (Gregarinomorpha n. nov.; Gregarinae Haeckel, 1866; Gregarinidea Lankester, 1885; grégarines des auteurs). Pp. 590-690 in P.-P. Grassé, ed. Traité de Zoologie (Vol. 1). Masson.
Hausmann, K., Hülsmann, N., and R. Radek. 2003. Protistology. 3rd completely revised edition. E. Schweizerbart’sche Buchhandlung (Nägele u. Obermiller) Stuttgart, 379pp.
Heintzelman, M. B. 2004. Actin and myosin in Gregarina polymorpha. Cell Motility and the Cytoskeleton 58: 83–95.
Kuriyama, R. et al. 2005. Dynamic organization of microtubules and microtubule-organizing centers during the sexual phase of a parasitic protozoan, Lecudina tuzetae (Gregarina, Apicomplexa). Cell Motility and the Cytoskeleton 62: 195–209.
Landers, S.C., and B. S Leander. 2005. Comparative surface morphology of marine coelomic gregarines (Apicomplexa, Urosporidae): Pterospora floridiensis and Pterospora schizosoma. Journal of Eukaryotic Microbiology 52: 23–30.
Leander, B. S. 2006. Ultrastructure of the archigregarine Selenidium vivax (Apicomplexa) - a dynamic parasite of sipunculid worms (Host: Phascolosoma agassizii). Marine Biology Research 2: 178–190.
Leander, B. S. 2007. Molecular phylogeny and ultrastructure of Selenidium serpulae (Apicomplexa, Archigregarinia) from the calcareous tubeworm Serpula vermicularis (Annelida, Polychaeta, Sabellida). Zoologica Scripta 36 (2): 213-227.
Leander, B. S. 2008. Marine gregarines: evolutionary prelude to the apicomplexan radiation? Trends in Parasitology 24(2): 60-67.
Leander, B. S., Clopton, R. E., and P. J. Keeling. 2003a. Phylogeny of gregarines (Apicomplexa) as inferred from small-subunit rDNA and beta-tubulin. International Journal of Systematic and Evolutionary Microbiology 53 (Pt 1): 345-354.
Leander, B. S., Harper, J. T., and P. J. Keeling. 2003b. Molecular phylogeny and surface morphology of marine aseptate gregarines (Apicomplexa): Selenidium spp. and Lecudina spp. Journal of Parasitology 89 (6): 1191-1205.
Leander, B. S. and P. J. Keeling. 2004. Early evolutionary history of dinoflagellates and apicomplexans (Alveolata) inferred from HSP90 and actin phylogenies. J. Phycol. 40:341-250.
Leander, B. S., Lloyd, S. A. J., Marshall, W., and S. C. Landers. 2006. Phylogeny of Marine Gregarines (Apicomplexa) -Pterospora, Lithocystis and Lankesteria - and the Origin(s) of Coelomic Parasitism. Protist 157 (1): 45-60.
Leong, K. L. H., Kaya, H. K., Yoshimura, M. A., and D. F. Frey. 1992. The occurrence and effect of a protozoan parasite Ophryocystis elektroscirrha (neogregarinida: Ohryocystidae) on overwintering monarch butterflies Danaus plexippus (Lepidoptera: Danaidae) from two California winter sites. Ecological Entomology 17(4): 338-342.
Levine, N. D. 1976. Revision and checklist of the species of the aseptate gregarine genus Lecudina. Transactions of the American Microscopical Society 95(4): 695-702.
Levine, N. D. 1977. Revision and checklist of the species (other than Lecudina) of the aseptate gregarine family Lecudinidae. Journal of Protozoology 24: 41–52.
Levine, N. D. 1988. Progress in taxonomy of the apicomplexan Protozoa. Journal of Protozoology 35(4): 518-520.
McFadden, G. I., Waller, R. F.,Reith, M., Munholland, J., and N. Lang-Unnasch. 1997. Plastids in apicomplexan parasites. Plant Systematics and Evolution (Supplementa) 11: 261-287.
McLaughlin, R. E., and J. Myers. 1970. Ophryocystis elektroscirrha sp. n. a neogregarine pathogen of the monarch butterfly Danaus plexippus (L.) and the Florida quenn butterfly Danaus gilippus berenice Cramer. Journal of Protozoology 17: 300-305.
Olsen, O.W. 1974. Animal parasites: Their life cycles and ecology. Dover Publications, Inc., New York.
Omoto, C.K., Toso, M., Tang, K and. Sibley, L.D., 2004. Expressed Sequence Tag (EST) analysis of Gregarine gametocyst development. Int. J. Parasit. 34:1265-1271.
Roychoudhury, S., Isawa, H., Hoshino, K., Sasaki, T., Saito, N., Sawabe, K., and Kobayashi, M. 2007. Comparison of the morphology of oocysts and the phylogenetic analysis of four Ascogregarina species (Eugregarinidae: Lecudinidae) as inferred from small subunit ribosomal DNA sequences. Parasitology International 56 (2): 113-118.
Rueckert, S., and B. S. Leander. 2008. Morphology and phylogenetic position of two novel marine gregarines (Apicomplexa, Eugregarinorida) from the intestines of North-eastern Pacific ascidians. Zoologica Scripta 37(6):637-645.
Schrével, J. 1971a. Observations biologique et ultrastructurales sur les Selenidiidae et leurs conséquences sur la systématique des grégarinomorphes. Journal of Protozoology. 18: 448–470.
Schrével, J. 1971b. Contribution a l’e´tude des Selenidiidae parasites d’annélides polychètes. II. Ultrastructure de quelques trophozoites. Protistologica 7: 101–130.
Stebbings, H. et al. (1974) Microtubules and movement in the archigregarine, Selenidium fallax. Cell Tissue Research 148: 331–345.
Toso, M. A., and C. K. Omoto. 2007. Gregarina niphandrodes may lack both a plastid genome and organelle. Journal of Eukaryotic Microbiology 54(1): 66.72.
Valles, S. M., and R. M. Pereira. 2003. Use of ribosomal DNA sequence data to characterize and detect a neogregarine pathogen of Solenopsis invicta (Hymenoptera: Formicidae). Journal of Invertebrate Pathology 84 (2): 114-118.
Vávra, J., and E. B. Small. 1969. Scanning electron microscopy of gregarines (Protozoa, Sporozoa) and its contribution to the theory of gregarine movement. Journal of Protozoology 16: 745–757.
Vivier, E. 1968. L’organization ultrastructurale corticale de la gregarine Lecudina pellucida: ses rapports avec l’alimentation et la locomotion. Journal of Protozoology 15: 230–246.
Vivier, E. and I. Desportes. 1990. Apicomplexa. In: Margulis, L., J.O. Corliss, M. Melkonian, and D.J. Chapman, eds. 1990. Handbook of the Protoctista; the structure, cultivation, habits and life histories of the eukaryotic microorganisms and their descendants exclusive of animals, plants and fungi. Jones and Bartlett Publishers. Boston. pp. 549-573.
Waller, R. F., and G. I. McFadden. 2005. The apicoplast: A review of the derived plastid of apicomplexan parasites. Current Issues in Molecular Biology 7: 57-80.
Wilson, R. J. M., and D. H. Williamson. 1997. Extrachromosomal DNA in the Apicomplexa. Microbiology and Molecular Biology Reviews 61: 1-16.
Wilson, R. J. M., Denny, P. W., Preiser, P. R., Rangachari, K., Roberts, K., Roy, A., Whyte, A., Strath, M., Moore, D. J., Moore, P. W., and Williamson, D. H. 1996. Complete gene mapof the plastid-like DNA of the malaria parasite Plasmodium falciparum. Journal of Molecular Biology 261: 155-172.
Xu, P., Widmer, G., Wang, Y., Ozaki, L., Alves, J., Serrano, M., Puiu, D., Manque, P., Akiyoshi, D., Mackey, A., Pearson, W., Dear, P., Bankier, A., Peterson, A., Abrahamsen, M., Kapur, V., Tzipori, S., and G. Buck. 2004. The genome of Cryptosporidium hominis. Nature 431: 1107-1112.
Zhu, G., Marchewka, M., and J. Keithly. 2000. Cryptosporidium parvum appears to lack a plastid genome. Microbiology 146: 315-321.
Title Illustrations

Scientific Name | Selenidium serpulae |
---|---|
Location | Bamfield, Vancouver Island, BC, Canada |
Specimen Condition | Live Specimen |
Identified By | Brian Leander |
Life Cycle Stage | Trophozoite |
Copyright |
© Brian S. Leander
![]() |
Scientific Name | Lecudina |
---|---|
Location | Vancouver, British Columbia |
Specimen Condition | Live Specimen |
Life Cycle Stage | Trophozoite |
Image Use |
![]() |
Copyright |
© Brian S. Leander
![]() |
Scientific Name | Pterospora schizosoma |
---|---|
Location | Bamfield, British Columbia |
Specimen Condition | Live Specimen |
Identified By | Brian Leander |
Life Cycle Stage | Trophozoites connected in syzygy |
Copyright |
© Brian S. Leander
![]() |
Scientific Name | Lankesteria cystodytae |
---|---|
Location | Bamfield, Vancouver Island, BC, Canada |
Specimen Condition | Live Specimen |
Life Cycle Stage | Trophozoite |
Image Use |
![]() |
Copyright |
©
![]() |
Scientific Name | Septate eugregarines |
---|---|
Specimen Condition | Live Specimen |
Image Use |
![]() |
Copyright |
©
![]() |
About This Page
This page is being developed as part of the Tree of Life Web Project Protist Diversity Workshop, co-sponsored by the Canadian Institute for Advanced Research (CIFAR) program in Integrated Microbial Biodiversity and the Tula Foundation.
University of British Columbia, Vancouver, British Columbia, Canada
Brian S. Leander
The University of British Columbia, Vancouver, British Columbia, Canada
Correspondence regarding this page should be directed to Sonja I. Rueckert at and Brian S. Leander at
Page copyright © 2011 and Brian S. Leander
All Rights Reserved.
- First online 23 September 2008
- Content changed 23 September 2008
Citing this page:
Rueckert, Sonja I. and Brian S. Leander. 2008. Gregarina http://tolweb.org/Gregarina/124806/2008.09.23 in The Tree of Life Web Project, http://tolweb.org/
. Gregarines. Version 23 September 2008.