One of the most spectacular forms of marine bioluminescence (often incorrectly called “marine phosphorescence“) is produced by dinoflagellates. In the sea, light emission by these unicellular organisms is mostly seen when cells are mechanically stimulated, at the surface of waves, in breakers, by swimming animals or humans or by vessels. Bioluminescence is only shown by a smaller number of dinoflagellate species. In some genera, this is a common property, e.g., in Pyrocystis (such as P. lunula, P. elegans, P. acuta, P. fusiformis and P. noctiluca), Lingulodinium (L. polyedrum, syn. Gonyaulax polyedra), Gonyaulax (G. spinifera), Pyrodinium (P. bahamense, P. phoneus) and Noctiluca (N. scintillans). Other genera contain both bioluminescent and nonluminescent species, e.g., Alexandrium (luminescent, e.g., A. affine, A. tamarense), Protoceratium (luminescent, e.g., P. reticulatum), Ceratium (luminescent: e.g., C. fusus). Sometimes, luminescent and nonluminescent strains were even found within one species, as is the case in Ceratocorys horrida (Valiadi et al. 2012).
Biochemical mechanism and genes involved in the luminescence reaction
In principle, light can be emitted in the course of nonenzymatic or enzymatic reactions. All systems that efficiently generate high, easily visible light intensities belong to the latter category, in which a substrate, mostly called luciferin, is oxidized by an enzyme, mostly referred to as luciferase (LCF). This terminology is also used in dinoflagellates. Luciferins and luciferases have evolved separately in various other taxa and are, with one exception, unrelated to those of dinoflagellates.
The fact that textbooks frequently use firefly bioluminescence as an example for explaining the biochemistry of light emission has contributed to the widely spread error that bioluminescence requires ATP as an energy source. The involvement of ATP is, in fact, a peculiarity of some insects including fireflies. The source of energy that leads to an excited molecular state of a luciferin intermediate from which light is emitted is, instead, the redox potential. In the majority of bioluminescent systems, including those of dinoflagellates, a reduced substrate molecule is oxidized, thereby transiently generating an excited state from which a photon is emitted. In dinoflagellates, the reduced substrate, luciferin, often referred to as LH2, is an open-chain tetrapyrrole (Fig. 1) (Hastings 1989, Nakamura et al. 1989). During oxidation by molecular oxygen (O2), an excited intermediate is formed, which is believed to be a hydroperoxyl adduct, from which light and water are released (Nakamura et al. 1989). The resulting oxidized luciferin (Lox) is different from another product generated by autoxidation of LH2 in a nonluminescent reaction. In thoroughly studied dinoflagellates, the light of the luminescent reaction has an emission maximum of 475 nm (Hastings 1996).
The dinoflagellate luciferin is rather unique under two aspects. First, an open-chain tetrapyrrole has been found to act as an LCF substrate, outside this group, only in a crustacean taxon, the euphausiids (Dunlap et al. 1980a, Nakamura et al. 1989). It differs from dinoflagellate luciferin only by a hydroxyl group attached to the methyl residue at pyrrole ring 2. In the euphausiids, a precursor possibly originating from dinoflagellates is believed to be taken up with the food. Second, the excited hydroperoxyl intermediate differs from all luminophores generated in other organisms, such as compounds carrying “active carbonyls“ produced in the decay of dioxetane structures, which are typical for many other luciferins.
The dinoflagellate luciferin shows a striking similarity to chlorophyll by possessing a five-membered ring attached to the third pyrrole. Although this may indicate a formation via chlorophyll catabolism, the biosynthetic pathway has not yet been identified. Dinoflagellate LH2 has been reported to be regenerated by an NAD(P)H-dependent luciferin reductase from Lox formed in the bioluminescent reaction (Fresneau et al. 1986, Fresneau and Arrio 1988).
Dinoflagellate luciferases (LCFs) of several species have been studied in detail and shown to profoundly differ from LCFs of other taxa (Bae and Hastings 1994). According to current knowledge from sequenced species, two types can be distinguished, one of them found in Lingulodinium, Alexandrium, Pyrocystis and Protoceratium, the other one in Noctiluca. The first type is characterized by the peculiarity of containing three enzymatically active catalytic domains in a single polypeptide chain (Li et al. 1997, Li and Hastings 1998, Okamoto et al. 2001, Morishita et al. 2002, Liu et al. 2004, Schultz et al. 2005). The catalytic domains form β-barrel pockets, to which the access of the substrate can be restricted by a three-helix bundle. The three catalytic domains are similar but not fully identical. In Lingulodinium polyedrum, a single lcf gene has been identified, which is present in multiple copies organized as tandem repeats. In Pyrocystis lunula, three different lcf genes can be distinguished, lcfA, lcfB and lcfC (Okamoto et al. 2001), which resemble in their basic organization that of L. polyedrum, with some specific deviations. The lcf gene of L. polyedrum does not contain any introns, whereas one intron is found in the lcfC gene of P. lunula. lcfA and lcfB genes are connected by a specific intergenic region. The full-length LCF protein of L. polyedrum as well as its three single domains exhibit a pronounced pH dependence, with highest activities at pH 6.3 and little activity at pH 8.0. As will be discussed below, this property is relevant to the triggering mechanism of bioluminescence in the intracellular luminescent microsources, the scintillons of L. polyedrum. The pH dependence has been related to the protonation of four histidine residues present in the N-terminal flanking regions of the respective catalytic domains (Schultz et al. 2005). These histidines do not participate in the catalytic process, but their protonation causes a substantial molecular motion which has been interpreted in terms of a conformational change in the three-helix bundle, thereby allowing the access of the substrate. Later, it was concluded from deletion experiments that also the C-terminal flanking regions of the catalytic domains are required for their enzymatic activity (Suzuki-Ogoh et al. 2008), but the structural mechanism remains to be identified.
The second type of an lcf gene that is found in Noctiluca scintillans encodes two functionally different domains (Liu and Hastings 2007). The first one, which is located in the N-terminal part of the LCF protein, exhibits homology to the catalytic domains of the other species discussed above. However, the second domain found in the C-terminal section is entirely different and shows, surprisingly, homology to the luciferin-binding protein (LBP) from L. polyedrum. This latter protein, which is another important player in the regulation of light emission, will be discussed next. The remarkable finding is that, in Noctiluca, one gene encodes two functionally different domains, which are present as separate genes in the other species. Noctiluca seems to be phylogenetically distant to the other luminescent species. Whether Noctiluca really represents a member of an earlier branch of primitive dinoflagellates (Fukuda and Endo 2008) should be judged with caution and is a matter of debate (Hoppenrath and Leander 2010). A certain distance would be consistent with the differences in the organization of lcf and lbp genes. However, with regard to the high number of nonluminescent dinoflagellate species, this may either lead to the assumption that bioluminescence is a relatively ancient characteristic of dinoflagellates or, alternately, that the lcf and lbp genes have been transduced within this group via horizontal gene transfer. The first possibility might be compatible with the existence of lcf pseudogenes in some nonluminescent species and strains (Valiadi et al. 2012), although pseudogenes may also arise from incomplete gene transfer.
Information on LBP has been mostly obtained from studies in L. polyedrum. Corresponding data are available from a cDNA library from Alexandrium tamarense (Hackett et al. 2005). In the L. polyedrum genome, two variants of the lbp gene are present, lbpα and lbpβ, which share about 86% sequence identity at both nucleotide and amino acid levels (Machabée et al. 1994, Tanikawa et al. 2004). Each of them is organized as tandem repeats, which are present in a high number of copies within the genome. In the species investigated, lbp genes do not contain introns and poly(A) attachment sites (Lee et al. 1993). The LBP protein exists as a dimer, in which each subunit binds one luciferin molecule (Hastings 1989). In the bioluminescent microsources, LBP is present in higher concentrations than LCF (Desjardins and Morse 1993). At night, LBP amounts to about 1% of total protein in L. polyedrum (Morse et al. 1989b). At moderate alkaline pH, LBP has a high affinity to luciferin, with an association constant (Ka) of about 5 × 107 M–1. LBP fulfills two important functions in the bioluminescent dinoflagellate cell. First, it protects the easily oxidizable luciferin from autoxidation. Second, it releases luciferin after acidification to below pH 7, most efficiently at pH 6.3 (Morse et al. 1989b). As will be outlined next, the acidification within the luminescent microsource represents the crucial step in the regulation of light emission in dinoflagellates. Whilst the role of LBP is relatively clear in L. polyedrum, and presumably also in related species, this is not the case with other dinoflagellates. LBP was reported to be absent from Pyrocystis lunula (Colepicolo et al. 1993). Since luminescence stimulation by acid is easily achieved in all Pyrocystis species tested, and since luciferin is clearly and tightly associated with the microsources, at least during night, the questions arise as to whether the effect of acidification is based on the pH-profile of LCF and by which mechanism luciferin is concentrated in the microsources.
Structures and function of bioluminescent microsources
The most thoroughly studied microsources are the scintillons of L. polyedrum (Fig. 2).
Fig. 2. Model of proton-stimulated bioluminescence of scintillons, the luminescent microsources of Lingulodinium polyedrum. A. Scintillons are cytoplasmic intrusions into acidic vacuoles and activated by conducted proton action potentials. Acidification of the interior of a scintillon by proton entry leads to light emission. B. Model of main components of the bioluminescent system and of processes that lead to LCF activity. Acidification causes release of luciferin from LBP (luciferin binding protein) and activation of LCF, which has three enzymatically active domains (cf. current text). C. Overview of the processes from opening of voltage-gated proton channels in the tonoplast/scintillon membrane to light emission. © Rüdiger Hardeland |
Microsources of other luminescent dinoflagellates can differ with regard to their structure and localization. Therefore, the term ‘scintillon‘ should be reserved for the luminescent organelles present in L. polyedrum and related species. Their peculiarity consists in their intracellular morphology. They are formed as cytoplasmic intrusions into acidic vacuoles (Fig. 2). In L. polyedrum, the acidic vacuoles are located in the periphery of the cell, as demonstrated by accumulation of the acidotropic vital stain, neutral red (Fig. 3).
In some Pyrocystis species, such as P. lunula, P. elegans and P. acuta, the acidic vacuoles and their microsources are concentrated in the center of the cell, whereas the considerably larger, almost spheric P. noctiluca contains a voluminous vacuole that makes up the major part of the cell. In P. noctiluca, the microsources are mostly located along several cytoplasmic strands adjacent to the vacuole (Fig. 4).

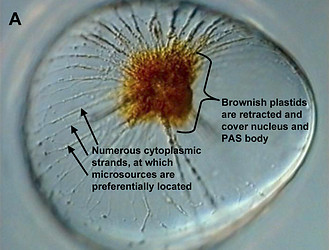
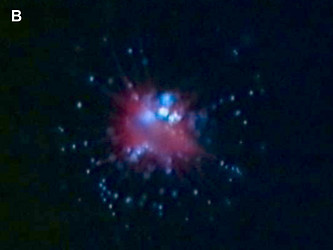
Fig. 4. The microsources of Pyrocystis noctiluca. A. A cell from dark phase with retracted chloroplasts, showing numerous cytoplasmic strands in the periphery. B. Autofluorescence picture showing chlorophyll fluorescence (red) of the retracted plastids and numerous microscources (blue luciferin fluorescence), which are mostly associated with the cytoplasmic strands. [From Behrmann and Hardeland 1999]. © Gudrun Behrmann & Rüdiger Hardeland
In L. polyedrum, the vacuole was shown to be mainly acidified by a V-type proton ATPase (Burkhardt et al. 1997). A small contribution of another, unknown proton pump may be possible, but carboanhydratase does not seem to be involved, because inhibitors of the latter enzyme did not block light emission or change the temporal bioluminescent patterns (Burkhardt et al. 1997). As a result of the intravacuolar acidification, a proton gradient is formed between vacuole and cytoplasm. The tonoplast of the acidic vacuole contains proton channels, which are crucial for the stimulation of luminescence. Recently, a voltage-gated proton channel has been described in the nonluminescent Karlodinium veneficum, whose Lingulodinium homolog is thought to be responsible for triggering light emission in L. polyedrum (Smith et al. 2011). From a functional point of view, proton channels have been concluded to be also present and, perhaps, enriched in the parts of the membrane that surround the cytoplasmic content of the scintillon (Fig. 2). In this scintillon membrane, LCF is concentrated as an integral membrane protein. LBP is present in particularly high amounts as a peripheral membrane protein attached to the cytoplasmic face of the scintillon membrane (Fig. 2). Under nonstimulated conditions, LBP is maximally loaded with luciferin. It can be assumed that also the luciferin reductase is present in the scintillons, which would allow a rapid regeneration of the LCF substrate LH2 from Lox.
Signal transduction mechanisms originating from mechanical or metabolic stimuli influence the proton transfer from the acidic vacuole to the cytoplasm. In fact, conducted proton action potentials that are initiated by such stimuli move along the tonoplast (Fritz et al. 1990). As soon as they reach a scintillon membrane, the interior of this structure is rapidly acidified. This results in two pH-dependent effects, as already indicated above, (i) a conformational change in the LCF protein, which removes the steric hindrance by the three-helix bundle and allows substrate access to the three active centers of LCF, and (ii) a conformational change in LBP, which considerably reduces its affinity to luciferin and causes its release, thereby making LH2 available to the LCF (Fig. 2). The spreading of the conducted H+ action potential leads to a series of sequential flashes emitted from different scintillons.
A spatiotemporal sequence of flashing was also observed in Pyrocystis noctiluca, after stimulation by citrate during the night phase (Fig. 5) (Behrmann and Hardeland 1999, Behrmann et al. 2001). This indicates, again, the spreading of a proton action potential, although the mechanism may be different from that of L. polyedrum, because LBP was reported to be absent from another Pyrocystis species, P. lunula (Colepicolo et al. 1993).
Mechanical shearing of cells represents a biologically important stimulus that initiates proton transfer and the subsequent processes described. A cellular shear receptor has been implicated in this mechanism, but molecular details and signal transduction remain to be elucidated in the luminescent species. In L. polyedrum, the involvement of Ca2+ mobilization from intracellular stores has been suggested (Dunlap et al. 1981). However, shear receptors are also present in nonluminescent species, in which mechanical stress can induce cell cycle arrest. In Crypthecodinium cohnii, caffeine was reported to stimulate Ca2+ release, whereas a ryanodine receptor antagonist, dantrolene, inhibited both the mechanically and the caffeine induced Ca2+ mobilization (Yeung et al. 2006). This could imply that the shear receptor resembles or is associated with a ryanodine-receptor type calcium channel rather than representing a typical mechanosensitive channel. However, the possibility remains that the shear receptor is upstream of the ryanodine-sensitive calcium channel. Whether these conclusions may be also valid for luminescent species and the initiation of light emission remains to be definitely demonstrated. In L. polyedrum, ruthenium red, a blocker of intracellular Ca2+ channels, inhibited mechanically stimulated bioluminescence (von Dassow and Latz 2002). Additional experiments using Ca2+ ionophores, chelators or digitonin may not be fully conclusive for reasons of unspecificity, but support the idea of calcium signaling. Although more direct evidence would be desired, it seems rather likely that Ca2+ triggers the conducted proton potential at the tonoplast of the acidic vacuole.
Proton transfer to the interior of the scintillon can be also induced by chemical stimuli. Numerous luminescence-stimulating toxins are known, but shall not be discussed here. In L. polyedrum, bioluminescence was strongly and selectively enhanced by epinephrine (Hardeland 1980, Balzer and Hardeland 1989), a biogenic amine detected in another dinoflagellate, but the mechanism was not elucidated in detail, except for the demonstration of proton transfer. Much more is known on an indoleamine that is physiologically present in dinoflagellates. L. polyedrum was the first organism outside the animals in which melatonin was detected (Poeggeler et al. 1991), previously known as the hormone of the vertebrate pineal gland. This molecule can attain concentrations by orders of magnitude higher than in vertebrates (Fuhrberg and Hardeland 1997, Fuhrberg et al. 1997), especially in response to moderate decreases in temperature. Elevated melatonin levels induce the deacetylation of this compound to 5-methoxytryptamine (Fuhrberg and Hardeland 1997, Fuhrberg et al. 1997, Hardeland et al. 2007). This deacetylated metabolite strongly stimulates bioluminescence, with demonstrable effects down to 2 × 10–8 M (Balzer and Hardeland 1991; Hardeland and Balzer 1993), by enhancing proton translocation from the acidic vacuole (Hardeland et al. 1995, Hardeland and Fuhrberg 1996, Hardeland 1999). Corresponding findings were obtained in other dinoflagellates, too (Hardeland 1999). 5-Methoxytryptamine was also shown to be required for a normal circadian glow peak. After blockade of the biosynthetic pathway by inhibiting tryptophan 5-hydroxylase, addition of melatonin or any other metabolite that leads to 5-methoxytryptamine restores the glow maximum, whereas those indolic metabolites that cannot be converted to 5-methoxytryptamine do not (Hardeland and Burkhardt 1997, Hardeland et al. 1999).
Flashing, glow, circadian rhythms and photoinhibition
Two modes of light emission are known from dinoflagellates, flashing and glow. The first one consists of discrete, discontinuous light emissions from individual microsources, which are activated by proton transfer according the spreading of the conducted H+ action potential. Glow represents a continuous emission of light at much lower intensities, which are usually invisible by the naked eye, but easily detected by sufficiently sensitive photomultipliers.
In ecological terms, flashing is the biologically meaningful type of luminescence. It is induced by shearing or damage, as caused by predators, which either touch the cells when collecting them or feed on them, or by water streams that occur in the vicinity of swimming organisms (Maldonado and Latz 2007), which may be either these predators or other animals including dolphins. Dinoflagellates are prey of especially small crustacians. The biological advantage of flashing is believed to attract second order predators, a phenomenon known under the term of “burglar alarm“ (Mensinger and Case 1992, Abrahams and Townsend 1993). In test systems set up in aquaria, it was shown that both fish (Mensinger and Case 1992) and a cephalopod (Sepia) (Fleisher and Case 1995) predate at night more efficiently on swimming crustaceans in the presence of luminescent dinoflagellates.
No such ecological meaning can be attributed to glow. This form of bioluminescence is particularly observed at the end of the night until dawn and is, thus, associated with the decay of microsources, which are, during the day, lower in number and less charged with the components of the luminescent system (Johnson et al. 1984, 1985, Johnson and Hastings 1986, Fritz et al. 1990). The dinoflagellate cell seems to spare the energy costs of the luminescent system during the day phase because of its inutility and decomposes most of the microsources.
The amount of glow relative to flashing strongly varies among dinoflagellate species. It is rather small in high-luminescence species of the genus Pyrocystis, but is well pronounced and easily measurable in Lingulodinium polyedrum. To chronobiologists the measurement of glow has been particularly valuable, because the glow peak is relatively narrow and allows determinations of the circadian phase with remarkably high precision (Krasnow et al. 1980, Dunlap et al. 1980b, Njus et al. 1981,Taylor and Hastings 1982, Taylor et al. 1982). The majority of such studies has been conducted in L. polyedrum. In these investigations, the spontaneously emitted bioluminescence has been measured, usually in populations of cells, but occasionally also in single cells (Krasnow et al. 1981). The patterns observed in these experiments strongly deviate from others in which light emission is stimulated, e.g., by mechanical stress (Biggley et al. 1969, Stolzenberg et al. 1995). Within a 24-h cycle, stimulated bioluminescence corresponds to the number and loading of microsources, i.e., the availability of luciferin, LCF and LBP. The maximum is typically found in the first half or middle of the night. Bioluminescence capacity can be determined by rapidly exhausting the luminescent system by externally adding acid. An example is given for single cells of Pyrocystis noctiluca in Fig. 6 (more details in: Hardeland and Nord 1984).
Measurements of spontaneous bioluminescence sometimes reveal differences in the proportions of flashing and glow in a single species (Fig. 7). The reasons are mostly unknown. They are usually not attributable to minor differences in the culture medium, nor to cell density. Cell-cell collisions did not substantially contribute to the relative amount of flashing, whereas a moderate effect because auf cell-vial collisions was detected (Krasnow et al. 1980). Notably, the intensities of spontaneous flashing are by orders of magnitude lower than those observed under stimulation.
Both types of luminescence, flashing and glow, exhibit pronounced diurnal rhythms. This is plausible because the ecological value of light emission by dinoflagellates is restricted to the night. At least in photosynthetic species, an endogenous and an exogenous component are superimposed and are both important in the natural environment. The endogenous periodicity persists as a free-running (nonsynchronized) rhythm for many days under constant conditions, especially in continuous dim light (Hastings 1960, Krasnow et al. 1980, 1981, Njus et al. 1981, Taylor and Hastings, 1982, Taylor et al. 1982, Johnson and Hastings 1986), in some species even for a few days in constant darkness (Hardeland 1982, Hardeland and Nord 1984). This rhythmicity is generated by circadian oscillators. Interestingly, the unicell L. polyedrum contains more than one, in parallel operating oscillators, which control different circadian functions (Roenneberg and Morse 1993, Morse et al. 1994). L. polyedrum is a classic chronobiological model organism that allows studies at the unicellular level, which extend from the earlier investigations on luminescence to numerous other cellular functions.
The exogenous component is known under the term of “photoinhibition“. Light given in any circadian phase is capable of suppressing light emission by the cells. This effect was reported to occur exclusively in photosynthetic species and to affect only mechanically stimulated bioluminescence (Esaias et al. 1973). This conclusion was drawn from the absence of photoinhibition in chemically induced luminescence. However, own experience in L. polyedrum and several Pyrocystis species reveals photoinhibition in spontaneous light emission, too (Hardeland, unpublished data). Upon transfer of cells into darkness, this effect fades relatively soon. Contrary to the earlier conclusion by Esaias et al. (1973), these observations speak against a direct interference of a photoinhibitory mechanism with the shear receptor and rather indicate a more general mode of suppression of luminescence. Notably, chemically induced light emission was applied in those earlier experiments by using acid or a high (0.4 M) concentration of Ca2+, treatments that strongly affect intracellular structure and integrity.
The observed occurrence of photoinhibition in phototrophs is, at least partially if not entirely, attributable to intracellular changes in plastidial morphology. The relationship between bioluminescence and chloroplast movements had already been stated by Swift and Taylor (1967) in Pyrocystis lunula. The biological relevance of these movements consists in the avoidance of light absorption by the plastidial pigments. Therefore, chloroplasts have to be retracted from the light emitting microsources. In lunate Pyrocystis species, such as P. lunula or P. elegans (Fig. 8), as well as in the spindle-like P. acuta, the plastids are shortened and become contracted outside the central light-emitting area of the cell (Swift and Taylor 1967, Töpperwien and Hardeland 1980, Hardeland et al. 1997, Seo and Fritz 2000). In some of these species, especially P. lunula, the chloroplasts are more or less retracted to the four cytoplasmic hornlets of the coccoid cell. In the almost spheric P. noctiluca, the plastids which are, in the light phase, expanded below the surface of the entire cell, are progressively retracted to the “cell pole“, a cytoplasmic center in which organelles like the nucleus and the PAS body (periodic acid-Schiff reagent body, lysosome-like organelle) are located (Hardeland and Nord 1984, Hardeland et al. 1997). Thereby, the plastids are withdrawn from the microsources positioned like strings of pearls at the cytoplasmic strands mentioned above (cf. Fig. 4). The extent of chloroplast movements is extraordinarily large in this species (Fig. 9). In L. polyedrum, the plastids are much less retracted, but correspondingly removed from the periphery (Rensing et al. 1980) where the acidic vacuoles and the scintillons are located.
The plastidial movements are subject to both circadian control and direct effects of light. In correspondence to the statements on photoinhibition, light given at different phases within the 24-h cycle causes plastid expansion relatively soon. Conversely, transfer to darkness favors plastid retraction, which has been documented in an individual P. lunula cell (Töpperwien and Hardeland 1980). This has to be distinguished in mechanistic terms from the endogenous, circadian rhythmicity of plastid movements. However, in the natural environment, the exogenous effects of light and darkness represent, in addition to circadian entrainment by the light/dark cycle, concomitant phenomena. The circadian rhythms of chloroplast position and morphology have been followed for several cycles in various Pyrocystis species (Töpperwien and Hardeland 1980, Hardeland and Nord 1984, Hardeland et al. 1997) and in L. polyedrum (Rensing et al. 1980).
Regulation mechanisms
Apart from the immediate effects on light emission as initiated by proton action potentials and local acidification, long-term regulation mechanisms have been described that aim to explain the circadian changes in the luminescence system. Differences between species have been partially described and are likely to occur. However, the only dinoflagellate species that has been thoroughly studied in this regard is to date L. polyedrum. In this organism, the three major molecular components of the luminescence system undergo circadian fluctuations of high amplitude, i.e., luciferin, LCF and LBP. A rhythm of luciferin content has been described (Bode et al. 1963), but can be also deduced from the varying loading and number of scintillons, as shown by autofluorescence imaging (Johnson and Hastings 1986, Fritz et al. 1990). In the absence of a known biosynthetic pathway, a rate limiting enzyme and its circadian control mechanism have, however, not been identified. A circadian rhythm of LCF activity (Johnson et al. 1984, Johnson and Hastings 1986) has been related to cycling amounts of LCF protein (Dunlap and Hastings 1981). Notably, no circadian changes in lcf mRNA concentration have been detected. Therefore, the rhythm in LCF protein was concluded to be caused by a translational control mechanism (Mittag et al. 1998, Rossini et al. 2003). The earlier assumption of control by turnover (Dunlap and Hastings 1981) does not seem to be at variance with this interpretation, but rather reflects an additional process that contributes to the complete cycle. In the course of scintillon decomposition as occurring in the phase of the glow maximum, LCF protein is likely degraded at enhanced rates. By contrast with L. polyedrum, two Pyrocystis species, P. lunula and P. noctiluca were reported to not exhibit circadian rhythms in LCF concentration (Knaust et al. 1998), findings that would be in line with the negligible amplitudes of circadian variations in glow activity, at very low glow intensities observed in these species. In Pyrocystis, LCF was assumed to undergo a circadian cycle of intracellular distribution rather than biosynthesis.
In L. polyedrum, the circadian rhythmicity of LBP has been studied in more detail. Again, most investigations report the absence of a rhythm in mRNA concentration, but high-amplitude periodicities in LBP synthesis and concentration (Morse et al. 1989a, 1990, Mittag and Hastings 1998). In a single study, rhythms of lbp mRNA concentration and intracellular distribution were described (Techel et al. 1996), but recent confirmation is missing. In search of mechanisms explaining the translational control of LBP synthesis, attempts have been made to identify proteins that bind to untranslated (utr) regions in the lbp mRNA. A loop structure in the 3’-utr sequence has been assumed to be responsible for the binding of an inhibitory protein, which prevents translation in circadian phases in which no de novo LBP synthesis is required. A protein with these properties was reportedly characterized and referred to in the literature as CCTR (circadian-controlled translational regulator) (Mittag et al. 1994), but these findings have later been questioned (Lapointe and Morse 2008). The translational regulation mechanism awaits further clarification.
Movie
Behrmann, G., Hardeland, R. 1999. Biolumineszenz und Tagesrhythmik bei Dinoflagellaten. [English version: Bioluminescence and diurnal rhythmicity in dinoflagellates.] IWF, Göttingen, C 2013.